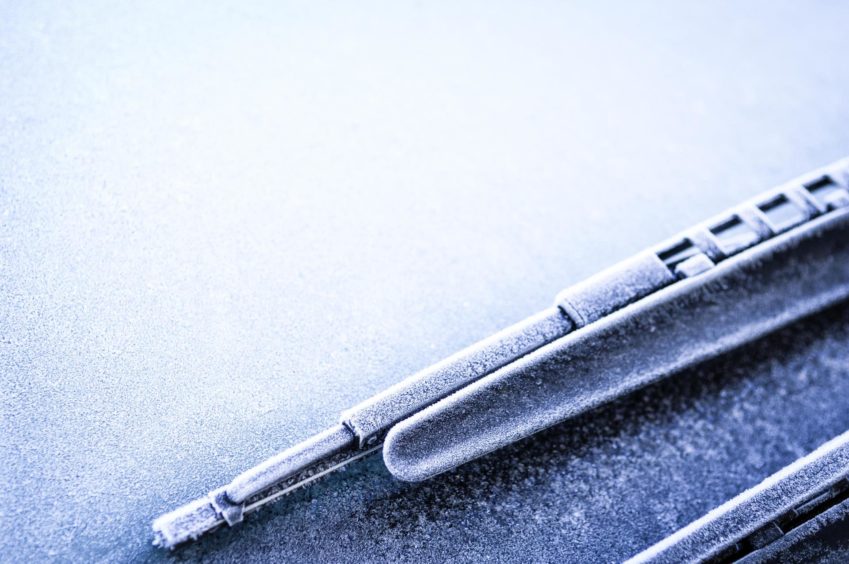
With the greatest and most urgent energy transition in human history accelerating, the quest for new technology solutions across multiple, diverse low carbon fronts is becoming ever more urgent.
While there’s a lot of blue skies work of the kind most readily associated with universities and government institutions going on; they are also heavily involved in finding new ways of making what we already have a lot more efficient.
Because of their huge importance to a cleaner, greener future, much of the research and, to some extent, development of future power generation and energy storage technologies, is concentrated into universities especially.
Due to its strategic importance, this attracts significant corporate and public purse funding, especially within the EU, Asia-Pac (particularly China), and the US.
In Part One published last month we concentrated on batteries because of their enormous energy storage criticality. Here in Part Two we catch up on some other low carbon R&D including:
· Sunlight, carbon dioxide and water into a carbon-neutral fuel – Cambridge University, UK
· High-power transparent solar cell development – Incheon National University, Korea.
· Wonder energy storage material – Lancaster University, UK
· Novel hydrolyser for extracting hydrogen from seawater – Penn State University, US
· Ammonia into Hydrogen – Northwestern University, US
Sunlight to fuel
The idea of developing a standalone device that converts sunlight, carbon dioxide and water into a carbon-neutral fuel might seem far-fetched, but is precisely what a team of scientists are doing at Cambridge.
It is seen as being a “significant step” towards achieving artificial photosynthesis – a process mimicking the ability of plants to convert sunlight into energy.
They have developed advanced ‘photosheet’ technology that converts sunlight, CO2 and water into oxygen and formic acid – a storable fuel that can be either be used directly or converted into hydrogen.
Basically it is a new way of converting CO2 into clean fuels. Moreover, the Cambridge scientists, working in collaboration with another group from Tokyo University, claim the process is scalable for deployment on energy ‘farms’. These would be similar to solar farms but produce clean fuel using sunlight and water rather than electricity.
Harvesting solar energy to convert CO2 into fuel is thought to be a promising way to reduce carbon emissions and transition away from fossil fuels.
However, it is challenging to produce such fuels without unwanted by-products.
“It’s been difficult to achieve artificial photosynthesis with a high degree of selectivity, so that you’re converting as much of the sunlight as possible into the fuel you want, rather than be left with a lot of waste,” says research team member Dr Qian Wang from Cambridge’s Department of Chemistry.
“In addition, storage of gaseous fuels and separation of by-products can be complicated.”
The team wants to get to the point where they can cleanly produce a liquid fuel that can also be easily stored and transported.
In 2019, a solar reactor based on an ‘artificial leaf’ design was developed. It too uses sunlight, CO2 and water to produce a fuel.
In the Cambridge case, the “photosheet” technology looks and behaves quite similarly to the artificial leaf but works in a different way and produces formic acid.
The sheets are made up of semiconductor powders, which can be prepared in large quantities easily and cost-effectively. It is considered more robust and produces clean fuel that is easier to store and shows potential for producing at scale.
The test unit is 20 sq.cm in size, but the researchers say that it should be relatively straightforward to up-scale to several square metres. In addition, the formic acid can be accumulated in solution, and be chemically converted into different types of fuel.
“We were surprised how well it worked in terms of its selectivity – it produced almost no by-products,” says Wang.
“Sometimes things don’t work as well as you expected, but this was a rare case where it actually worked better.”
Transparent PV
Staying with the sun, that ultimate source of energy for us all. Little wonder that there is a huge effort worldwide to advance photovoltaics technology, which has come on in leaps and bounds over the past decade especially.
Now, Korean scientists have put forward an innovative design for the development of a high-power transparent solar cell that might typically be integrated into windows, vehicles, cellphone screens, and other everyday products.
But for this to happen, PV technology itself has to be see-through, something that a team of scientists at the Incheon National University – Republic of Korea have achieved.
Their “transparent photovoltaic” (TPV) is basically a see-through variant of the traditional solar cell.
Unlike the conventionally dark, opaque solar cells (which absorb visible light), TPVs make use of the “invisible” light that falls in the ultraviolet (UV) range.
Conventional solar cells can be either “wet type” (solution based) or “dry type” (made up of metal-oxide semiconductors). Of these, dry-type solar cells have a slight edge over the wet-type ones: they are more reliable, eco-friendly, and cost-effective. Moreover, metal-oxides are well-suited to make use of the UV light.
The Korean team have come up with a novel design for a metal-oxide-based TPV device, which involves inserting an ultra-thin layer of silicon (Si) between two transparent metal-oxide semiconductors.
Prof Joondong Kim, who is leading the work says: “Our aim was to devise a high-power-producing transparent solar cell, by embedding an ultra-thin film of amorphous Si between zinc oxide and nickel oxide.”
This novel design apparently offers three major advantages.
· First, it allows for the utilisation of longer-wavelength light (as opposed to bare TPVs).
· Second, it results in efficient photon collection.
· Third, it allows for the faster transport of charged particles to the electrodes.
Moreover, the design offers the potential to generate electricity even under low-light situations, such as on cloudy or rainy days) and, during trials, it was used to drive a DC fan.
The Koreans believe their trail-blazing technology will soon find a market.
Its commercial potential is considered enormous and across a broad front: from glass buildings to mobile devices like electric cars, smartphones, and sensors.
“Not just this,” says Prof Kim, “the team is excited to take their design to the next level, by using innovative materials such as 2D semiconductors, nanocrystals of metal-oxides, and sulphide semiconductors.”
Now let’s switch from harvesting solar energy to generate electricity to harvesting its heat instead.
Wonder heat storage
It seems a team of scientist at Lancaster University have come up with a wonder material that may one day play a pivotal role the provision of effective, economical heat storage for on-demand use.
Whilst working on a crystalline material, they discovered that it has properties that allow it to capture energy from the sun. The energy can be stored for several months at room temperature, and later released on demand in the form of heat.
With further development, the Lancaster team’s view is that such materials have the potential to capture solar energy during the summer months and store it for use in winter, perhaps as an environmentally-responsible supplement to conventional heating in houses and offices.
It could be produced as a thin coating and applied to the surface of buildings, or used on the windscreens of cars where the stored heat could be used to de-ice on freezing winter mornings.
The material is based on a type of “metal-organic framework”’ (MOF). This comprises a network of metal ions linked by carbon-based molecules to form 3D structures.
A key property of MOFs is that they are porous, meaning that they can form composite materials by hosting other small molecules within their structures.
The Lancaster team set out to discover if a MOF composite, previously prepared by a separate research team at Kyoto University in Japan and known as ‘DMOF1’, can be used to store energy — something not previously researched.
The MOF pores were loaded with molecules of azobenzene — a compound that strongly absorbs light. These molecules act as photo-switches, which are a type of ‘molecular machine’ that can change shape when an external stimulus, such as light or heat, is applied.
In tests, the researchers exposed the material to UV light, which causes the azobenzene molecules to change shape to a strained configuration inside the MOF pores.
This process stores the energy in a similar way to the potential energy of a bent spring. Importantly, the narrow MOF pores trap the azobenzene molecules in their strained shape, meaning that the potential energy can be stored for long periods of time at room temperature.
The energy is released again when external heat is applied as a trigger to ‘switch’ its state, and this release can be very quick — a bit like a spring snapping back straight. This provides a heat boost which could be used to warm other materials of devices.
Further tests showed the material was able to store the energy for at least four months.
Although the results were promising for this material’s ability to store energy for long periods of time, its energy density was modest.
Next steps are to research other MOF structures as well as alternative types of crystalline materials with greater energy storage potential.
The research is supported by the Leverhulme Trust.
Seawater electrolyzer
Electrolysers have been around a long time, but using freshwater. Trying to extract (green) hydrogen from seawater has been more challenging.
However, it seems that is about to change as scientists/engineers at Penn State University in the US have successfully integrated water purification technology into a new proof-of-concept design for a sea water electrolyser.
This is excellent news for those who dream of harnessing surplus power from offshore wind farms to power electrolysers and produce commercial quantities of hydrogen.
Until the Penn team’s breakthrough, unless it is first desalinated prior to passing through an electrolyser, the chloride ions present in seawater turn into toxic chlorine gas, which degrades the equipment and seeps into the environment.
To prevent this, the researchers inserted a thin, semipermeable membrane, originally developed for purifying water in the reverse osmosis (RO) treatment process. The RO membrane replaced the ion-exchange membrane commonly used in electrolysers.
The idea behind RO is that you put a really high pressure on the water and push it through the membrane and keep the chloride ions behind.
In an electrolyser, seawater would no longer be pushed through the RO membrane, but contained by it.
A membrane is used to help separate the reactions that occur when hydrolyser electrodes are excited.
When the power is turned on, water molecules start splitting at the anode (positive side), releasing hydrogen ions called protons and creating oxygen gas.
The protons then pass through the membrane and combine with electrons at the cathode to form hydrogen gas.
With the RO membrane inserted, seawater is kept on the cathode (negative) side and the chloride ions are too big to pass through the membrane and reach the anode, averting the production of chlorine gas.
But in seawater splitting, other salts are intentionally dissolved in the water to help make it conductive. The ion-exchange membrane, which filters ions by electrical charge, allows salt ions to pass through. The RO membrane does not.
the Penn team have tested two commercially available RO membranes and two cation-exchange membranes, a type of ion-exchange membrane that allows the movement of all positively charged ions in the system.
Recently, the researchers received a $300,000 grant from the US National Science Foundation (NSF) to continue investigating sea water electrolysis.
Meanwhile, Saudi Arabia intends to invest in a $5billion hydrogen facility based on seawater feedstock. But it has to be desalinated before hydrolysing and that’s the kind of expensive intermediate step that seawater hydrolysers would eliminate.
Ammonia to hydrogen
American scientists have developed an apparently “highly effective”, environmentally responsible way of converting ammonia into hydrogen.
It is claimed to be a major step towards enabling a zero-pollution, hydrogen-fuelled economy.
The idea of using ammonia as a carrier for hydrogen delivery has gained traction in recent years because it is much easier to liquify than hydrogen and so convenient to store and transport.
The breakthrough achieved at Northwestern University, Illinois disposes of several existing barriers to the production of clean hydrogen from ammonia.
“The bane for hydrogen fuel cells has been the lack of delivery infrastructure,” says team member Professor Sossina Haile.
“It’s difficult and expensive to transport hydrogen, but an extensive ammonia delivery system already exists. There are pipelines for it. We deliver lots of ammonia all over the world for fertiliser.
“If you give us ammonia, the electro-chemical systems we developed can convert that ammonia to fuel-cell-ready, clean hydrogen on-site at any scale.”
Haile and her research team report they are able to conduct the ammonia-to-hydrogen conversion using renewable electricity instead of fossil-fuelled thermal energy because the process functions at much lower temperatures than traditional methods (250 degC as opposed to 500-600 degC.
Moreover, the new technique generates pure hydrogen that does not need to be separated from any unreacted ammonia or other products.
And, the process is efficient because all of the electrical current supplied to the device directly produces hydrogen, without any loss to parasitic reactions.
Because the hydrogen produced is pure, it can be directly pressurised for high-density storage by simply ramping up the electrical power.
The Northwestern team built a test rig that included a proton-conducting membrane and integrated it with an ammonia-splitting catalyst.
“The ammonia first encounters the catalyst that splits it into nitrogen and hydrogen,” Haile says.
“That hydrogen gets immediately converted into protons, which are then electrically driven across the proton-conducting membrane in our electrochemical cell.
“By continually pulling off the hydrogen, we drive the reaction to go further than it would otherwise. This is known as Le Chatelier’s principle.
“ By removing one of the products of the ammonia-splitting reaction — namely the hydrogen — we push the reaction forward, beyond what the ammonia-splitting catalyst can do alone.”
The hydrogen generated from the ammonia splitting then can be used in a fuel cell and Haile predicts that the new technology could be especially transformative in the transportation sector and out-run the battery alternative.
Why? The answer is simple.
Converting ammonia to hydrogen on-site and in a distributed way would enable one to drive into a forecourt and quickly replenish.
There’s also a growing interest in hydrogen fuel cells for the aviation industry because batteries are so heavy.